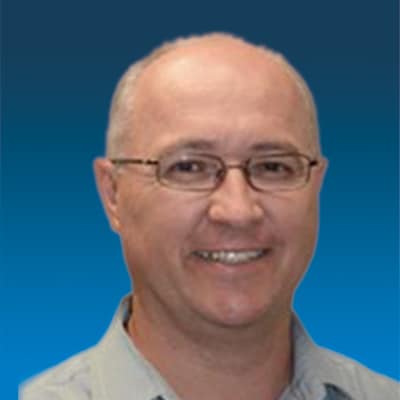
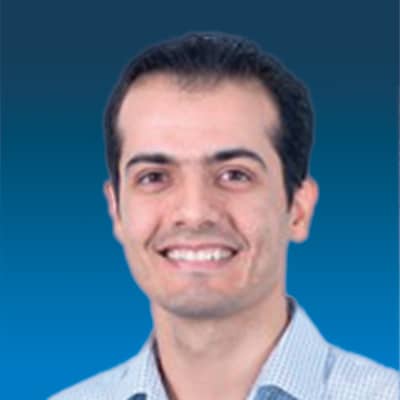
Djino Pirsic & Hesam Marzooghi
The make-up and operation of power systems (whether at a grid level or for smaller islanded systems) is becoming more complex with the increasing penetration of diverse intermittent renewable energy sources. As the amount of available wind and sunlight can vary, these resources are inherently intermittent which can make continuous power generation from these sources unreliable. To cover the variability in generation output, a source of energy must be available to offset the imbalance and provide power during low outputs and absorb power during high outputs of such intermittent renewable energy sources. This trend is leading to significant changes in how electricity is being generated and how networks need to be managed. In the last ten years, Battery Energy Storage Systems (BESS) have proven to be a technology enabler, allowing greater penetration of intermittent renewable inverter-based resources (IBR) into power systems including islanded grids or micro-grids. Apart from the inherent intermittency of IBR renewable energy, many studies, and experiences of network operators, are showing that the increasing integration of IBRs has been displacing system strength and inertia in the power systems, historically provided by rotating synchronous machines. It is expected more advanced BESS utilizing grid-forming technology will assist future power systems by providing system strength and virtual inertia.
Inverter power electronics developments
In recent years, the continuing market demand across many sectors has driven developments in inverter equipment (power electronics capability and modularisation, in particular) and heavy investment and R&D in battery technology have resulted in the faster and significantly more economical deployment of BESS installations. There are now more often reports about emerging and superior battery chemistries and capabilities (such as Lithium-sulphur, Lithium-air, Sodium-Ion, molten metal and solid-state batteries) however, equally as significant, developments have been occurring with inverter power electronics, energy management systems, battery management systems and in the way that these systems are integrated, housed and installed.
Recent commercial developments of high-power Wide Band Gap (WBG) devices allow the solid-state power switches (the inverter’s main semiconductor switching elements) to operate at higher voltages, temperatures and frequencies. Silicon carbide (SiC) and gallium nitride (GaN) power semiconductor substrates (both are WBG technologies) have been in commercial development for about ten years and are now being commercialized in next-generation PV and energy storage inverter power modules as well as in electric vehicle applications. WBG technology inverters have benefits such as reduced size and weight of components, ability to operate at higher voltages and temperatures, and achieving > 98% efficiencies which are 50% better than previous generation inverters and 20% better than the latest silicon semiconductor technology[i]. Figure 1 shows the position of WBG devices in relation to traditional silicon semiconductors in terms of output power and switching frequency.
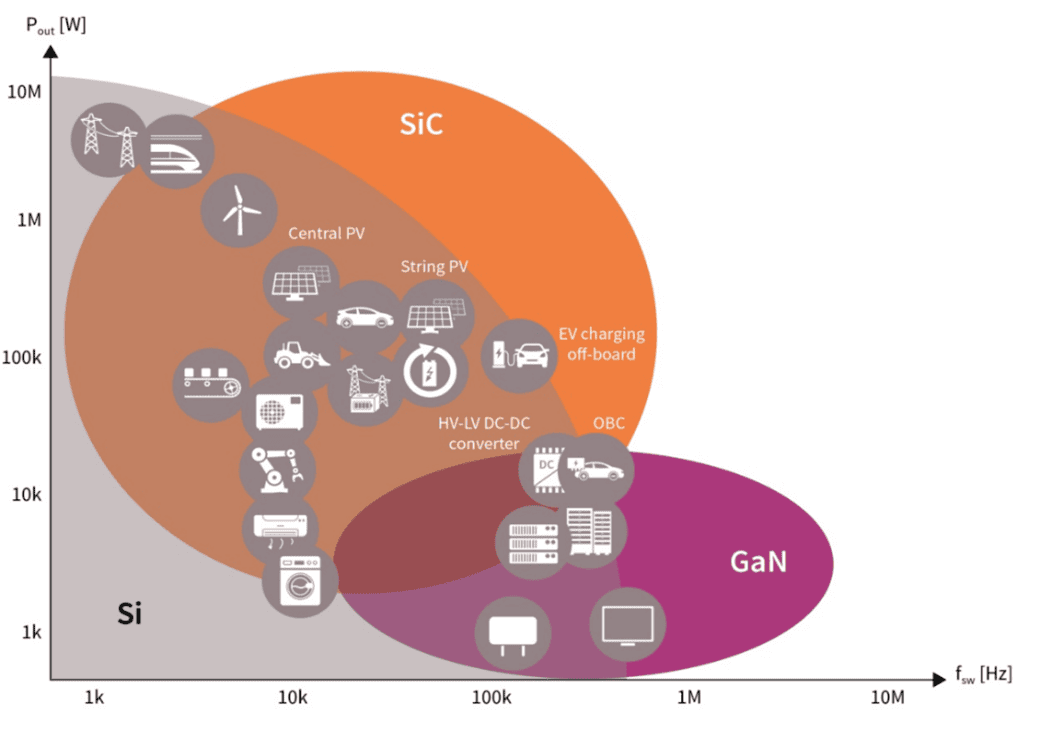
Figure 1: A comparison of WBG technology to Silicon in terms of frequency and power applications. ii Wide Bandgap Semiconductors Applications. ©Infineon Technologies AG www.infineon.com
It is expected that further development of WBG devices will result in higher performance inverters for PV and BESS applications. The advancement for BESS, however, is not only limited to better power switches. With massive R&D efforts in battery technology and chemistries, their capabilities have improved significantly in the past decade.
Battery developments
Lithium-Ion Batteries (LIB) have seen rapidly increasing development and commercial take-up for both mobile and stationary battery applications. LIB chemistries are quite diverse due to the outcomes of many research and development paths, driven by unprecedented demand. Figure 2 compares the most common commercial battery chemistries used for large-scale energy storage in terms of cost, efficiency, thermal stability, maturity and ratings. There are some viable alternatives to LIBs for energy storage using various technologies. Some have been commercialized for decades, capable and well-understood technologies such as pumped hydro and flywheels, but in comparison, some emerging technologies have not been widely adopted at this time for large-scale storage and conversion back to electrical power, such as hydrogen gas and sodium-ion batteries. Figure 3 shows the relative capabilities of viable storage methods.
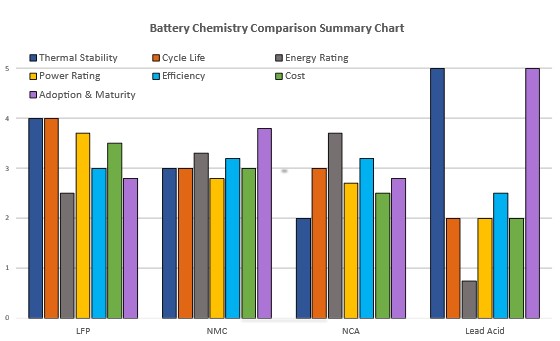
Figure 2: Battery cell performance comparison chart for common batteries used for large-scale energy storage ) LFP: Lithium Iron Phosphate, NMC: Lithium Nickel Manganese and Cobalt Oxide, NCA: Lithium Nickel Cobalt and Aluminium, Lead Acid: Lead Acid Absorbent Glass Mat / Gel [iii]
Grid–following inverters
Most inverters that are currently installed for IBR renewable generation are of the grid-following type (although grid-forming inverters may become more common – refer to next section). They are commonly referred to as current source inverters which require, by design, other generators in the network (usually synchronous generators) to provide a frequency reference and to regulate the network voltage, as shown in Figure 4. The grid-following inverters will synchronize to and “follow” the grid. They essentially feed power into the network (by controlling current) and can support network voltage and power factor to an extent, but they do not regulate the network and they may shut down if the network voltage or frequency is outside of the inverter’s limits.
Grid-following inverters typically act as a current source on the network and inject real (P) and reactive (Q) power into the network within the inverters’ power and stability limits. Grid-following inverters historically used current source DC supplies, where input current is relatively constant (however this is not a strict rule as some modern inverters can act as voltage or current sources with the same hardware) they are relatively slow to respond in adjusting their output power and this can be detrimental to network stability. They can be tuned to act faster to respond in adjusting their output power, however, this is seen to be detrimental to network stability in weak grids. Grid-following inverters cannot operate effectively or start without the network voltage and frequency being within normal limits. Their output voltage can vary depending on the load impedance they are connected to, and they cannot perform a black start of a network.
From a stability point of view, too many grid-following inverters in any network may lead to lower than acceptable network strength, increasing the likelihood of stability problems during network disturbances, which as a result may prevent the addition of more wind and solar farms to the affected parts of the network. Historically, relatively expensive measures such as installing synchronous condensers (synchronous rotating machines or syncons) have been used to compensate for this problem.
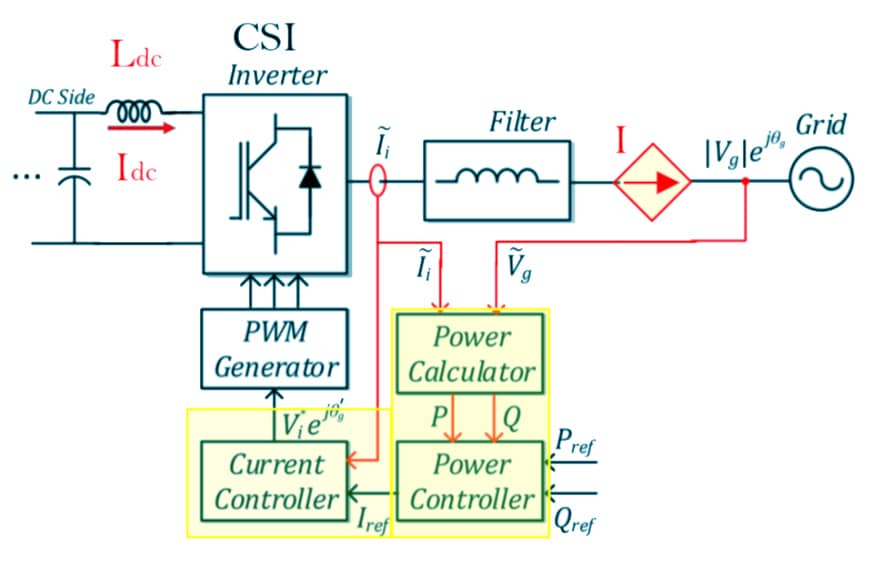
Figure 4: Simplified diagram of a Current Source Inverter (CSI) adapted from [v]
Grid–forming inverters, solving the inertia issue?
Grid-forming inverters (commonly referred to as voltage source inverters) on the other hand are a class of inverters that can provide their own frequency reference and can regulate their output voltage, and the network voltage if they are sufficiently large, as illustrated in Figure 5.
Grid-forming inverters can provide a source of system strength (with virtual inertia) to assist with stabilizing network voltage and frequency, essentially providing the “electronic” equivalent of rotating machine inertia. They can respond almost instantaneously to network disturbance, adjusting their power output without external control to provide a stabilizing function to the network by delivering damping and fault ride through capability.
Grid-forming inverters act like a voltage source on the network. They have internal references for output voltage and frequency, and they output power to maintain a network voltage and frequency (within the inverters’ power and stability limits). This is essentially equivalent to grid-connected rotating synchronous generators. Voltage source inverters’ control characteristics allow direct control of voltage and frequency. During network contingencies, the inverter will increase or decrease its output power almost instantaneously to maintain its terminal voltage and frequency. This is a similar characteristic to grid-connected rotating synchronous generators. Grid-forming inverters have the advantage of rapid response and the ability to perform a black start of a network as long as the load on the network does not exceed the overload capacity of the inverter.
The useful capabilities of advanced grid-forming inverters are now being understood by network operators and regulators. Incorporating the appropriate quantity of grid-following inverters into strategic parts of networks is seen as a top priority to allow greater penetration of IBR and to effectively compensate for the diminishing capacity of rotating synchronous generators in future power systems.[vii]
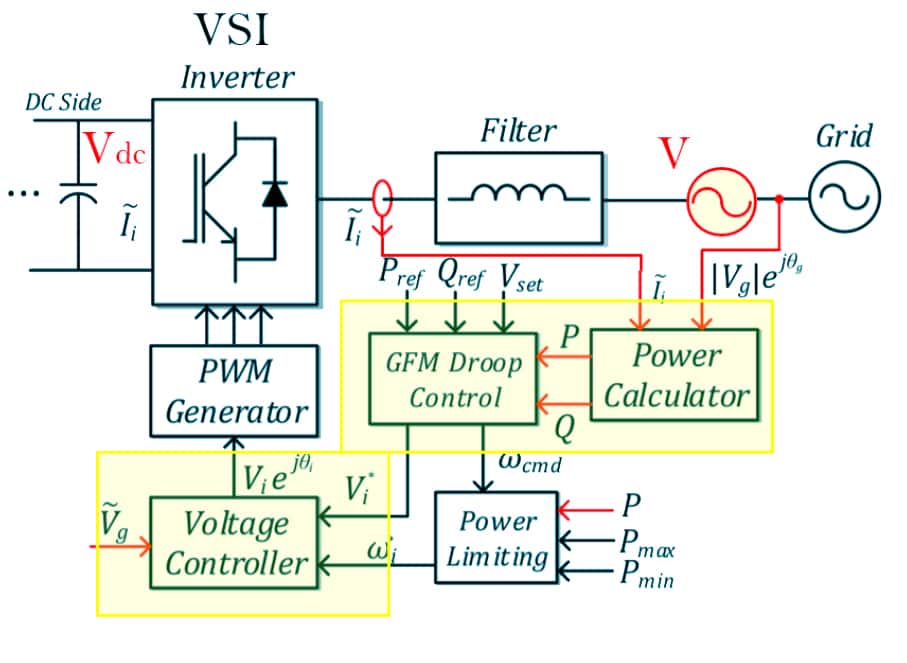
Figure 5: Simplified diagram of a Voltage Source Inverter (VSI) adapted from [v]
References
[i] S. Bala, G. Demetriades, F. Canales, A. Bianchi and F. Tombelli, “Applications for Wide Band Gap devices, Perspectives from ABB,” ABB, https://www.freedm.ncsu.edu/wp-content/uploads/2019/04/Bala-ABB-WBG-Applications.pdf, 2019.
[ii] Ref: Wide Bandgap Semiconductors Applications. ©Infineon Technologies AG https://www.infineon.com/cms/en/product/technology/wide-bandgap-semiconductors-sic-gan/
[iii] PSC internal – Battery Technology – Overview of rechargeable batteries for grid-scale applications.
Lithium-Ion Battery Chemistries by John T. Warner, Elsevier Publications, 2019, ISBN: 978-0-12-814778-8
Battery University, Types of Lithium-ion Batteries, https://batteryuniversity.com/article/bu-205-types-of-lithium-ion
Batteries for Electric Cars – Challenges, Opportunities and the Outlook for 2020. The Boston Consulting Group, January 1, 2010, https://www.ourenergypolicy.org/resources/batteries-for-electric-cars-challenges-opportunities-and-the-outlook-to-2020/
[iv] DOE/EPRI 2013 Electricity Storage Handbook in Collaboration with NRECA, SANDIA REPORT SAND2013-5131 Unlimited Release July 2013. Abbas A. Akhil, Georgianne Huff, Aileen B. Currier, Benjamin C. Kaun, Dan M. Rastler, Stella Bingqing Chen, Andrew L. Cotter, Dale T. Bradshaw, and William D. Gauntlett. Sandia National Laboratories Albuquerque, New Mexico. Available at: https://www.sandia.gov/app/uploads/sites/163/2021/09/SAND2013-5131.pdf
[v] IEEE Lasseter et al, “Grid-Forming Inverters: A Critical Asset for the Power Grid,” IEEE, 2020.
[vi] “Energy Systems and Storage Lab,” Centre for Renewable Energy Systems Technology (CREST) Loughborough University, 2017. [Online]. Available: http://www.eseslab.com/index. [Accessed April 2022].
[vii] S. Cherevatskiy, S. Sproul and S. Zabihi, “Grid Forming Energy Storage System addresses challenges of grids with high penetration of renewables (A case study),” 2020. [Online]. Available: https://www.electranet.com.au/wp-content/uploads/2021/02/CIGRE48-Grid-Forming-BESS-Case-Study-August-2020.pdf. [Accessed 03 2020].
The role of BESS in future power systems-Part 2 – PSC Consulting